Difference between revisions of "Interplanetary SmallSats"
(60 intermediate revisions by 3 users not shown) | |||
Line 1: | Line 1: | ||
<html> | |||
<script src="https://fred-wang.github.io/TeXZilla/TeXZilla-min.js"></script> | |||
<script src="https://fred-wang.github.io/TeXZilla/examples/customElement.js"></script> | |||
</html> | |||
=Technology Roadmap Sections and Deliverables= | =Technology Roadmap Sections and Deliverables= | ||
Line 39: | Line 44: | ||
[[Image:4SMS_FOM.png|1200px]] | [[Image:4SMS_FOM.png|1200px]] | ||
Data rate, power consumption, and mass are critical parameters for CubeSats, impacting mission success. Data rates for CubeSats have steadily improved from around 1200 bps in the 1990s to 10 Mbps today, mainly due to advances in RF transceivers, but are limited by the Shannon-Hartley theorem, which considers bandwidth and signal-to-noise ratio. Power consumption has also decreased from 5 watts in the 1990s to 3 watts in recent systems, thanks to energy-efficient designs, though CubeSats face constraints due to limited solar energy and battery storage. Mass reduction, achieved through miniaturization and material advances, has been modeled with a logistic function, showing rapid decreases in recent decades but approaching a theoretical limit due to essential components and operational durability in space. | |||
A logistic function is used to model satellite mass reduction, where parameters include a theoretical minimum (L), midpoint year of rapid reduction (t₀), and growth rate (k). The curve indicates initial high mass, followed by rapid decreases, then a plateau as technological limits are approached. | |||
==Alignment with Company Strategic Drivers== | ==Alignment with Company Strategic Drivers== | ||
== | Roughly every 3-5 years, NASA publishes a "Strategic Plan" intended to outline the key drivers behind their research, exploration, and discovery activities. The most recent version of this report is the [https://www.nasa.gov/wp-content/uploads/2023/09/fy-22-strategic-plan-1.pdf?emrc=ff1a1e NASA Strategic Plan 2022]. Interplanetary SmallSats support Strategic Objectives 1.2 (Understand the Sun, solar system, and universe) and 3.1 (Innovate and advance transformational space technologies). Fundamentally, NASA cares about its ability to gain a deeper scientific understanding of its exploration destinations. While there is no direct mention of SmallSat technologies in the Strategic Plan, it is clear that they value both more scientific data and more remote destinations. As a result, '''uplink data rate''' and '''power efficiency''' will be the key FOMs to advance NASA's goals. | ||
MarCO, the Mars CubeSat mission (2018), had spacecraft with power efficiency of 2.6 W/kg near Earth and 1.26 W/kg near Mars. This supported a data rate of 8 kilobytes per seconds (kbps) [https://www.jpl.nasa.gov/news/press_kits/insight/launch/appendix/mars-cube-one/ (source)]. The Lunar Flashlight project from a few years later nearly doubled the efficiency of MarCO's peak, operating at ~55W and only weighing 0.5 kg more than each MarCO satellite [https://ntrs.nasa.gov/api/citations/20190004974/downloads/20190004974.pdf (source)]. Its efficiency at its end-of-life was about 3.93 W/kg. The uplink data rate, however, was the same as MarCO's: 8 kbps. Note that this is not a completely fair comparison, as Mars is usually over 500 times farther away than the Moon. | |||
These two examples demonstrate that there is room for improvement in both FOM's. | |||
We can look to Earth-orbiting satellites for examples of what is technologically feasible. Earth-orbiting large satellite readily achieve uplink data rates in the tens of megabytes range. State-of-the-art Earth-orbiting small satellites claim they can achieve somewhere in the [https://www.aac-clyde.space/what-we-do/space-products-components/communications/cubecat hundreds of kbps]. Power efficiency for Earth-orbiting small satellites typically produce around [https://doi.org/10.1016/B978-0-7506-1162-6.50057-5 10 W/kg]. We believe these are ambitious, but achievable goals. Specifically, over the next decade as NASA prepares for increased human exploration activity, we believe it should aim to produce interplanetary SmallSats with uplink data rates in the '''~50 kbps range''' and power efficiency in the '''10 W/kg range'''. | |||
== Positioning of Company vs. Competition == | |||
The table below summarizes several notable interplanetary satellite missions and related efforts by NASA and other organizations to assess the competitive landscape for interplanetary small satellites. These missions are selected based on public data and illustrate the progression in uplink data rates, power efficiency, and payload capacities. They demonstrate evolving figures of merit (FOM) targets and underscore the unique challenges interplanetary satellites face compared to Earth-orbiting counterparts (NASA, 2022; JPL, 2018). | |||
[[File:Interplanetary Satellites Competitive Analysis.png|800px|thumb|center|The table summarizes notable interplanetary satellite missions, showing progression in FOMs and unique challenges faced by interplanetary satellites compared to Earth-orbiting counterparts (NASA, 2022; JPL, 2018).]] | |||
Interplanetary small satellites often face steep requirements in power efficiency and data transmission compared to Earth-orbiting satellites, as shown by comparing MarCO’s low data rate with that of typical Earth-orbiting satellites achieving over 100 kbps. In recent projects like Lunar Flashlight and Lunar Trailblazer, we see improvements in power efficiency, with Flashlight achieving nearly double MarCO’s peak efficiency. However, most missions struggle with maintaining high data rates across vast distances, given the limitations in miniaturized radio technology for deep space (NASA, 2022; ESA, 2020). | |||
Competitive FOM targets demonstrate that despite growing advancements, NASA’s interplanetary small satellites lag behind some Earth-orbiting satellites in terms of uplink data rates and power efficiency. While Earth-orbiting satellites can reach power efficiencies of 10 W/kg, interplanetary missions rarely achieve more than 4-5 W/kg, and uplink rates remain around 10 kbps or lower (ESA, 2020). As illustrated in the table, other space agencies, such as ESA, are pursuing interplanetary small satellites with similar limitations. This competitive analysis highlights how constraints unique to deep space missions slow the attainment of ambitious FOM targets seen in NASA’s Strategic Objective 3.1. | |||
<html> | |||
<script src="https://fred-wang.github.io/TeXZilla/TeXZilla-min.js"></script> | |||
<script src="https://fred-wang.github.io/TeXZilla/examples/customElement.js"></script> | |||
</html> | |||
==Technical Model== | ==Technical Model== | ||
===Sensitivity Analysis=== | |||
'''Energy Bit per Noise Spectral Density''' | |||
The first equation we analyzed is the energy bit per noise spectral density given by: | |||
<html> | |||
<la-tex>\frac{E_b}{N_0}=\frac{P_t L_{line} G_t L_{space} L_{atm} G_r}{k T_{sys} R}</la-tex> | |||
<br> | |||
Where <la-tex>L_{space} = \frac{4 r^2}{\lambda^2}</la-tex> and is the free space path loss. The other unknowns are listed below with some general values for MarCO, a Mars CubeSat mission launched in 2018. | |||
</html> | |||
{| class="wikitable" | |||
! Variable !! Quantity Represented !! Value !! Unit !! Source | |||
|- | |||
| <html><la-tex>P_t</la-tex></html> || Power transmitted || 17 || W || [https://www.jpl.nasa.gov/news/press_kits/insight/launch/appendix/mars-cube-one/ JPL] | |||
|- | |||
| <html><la-tex>L_{line}</la-tex></html> || Line loss || 0.6966 || unitless || [https://ieeexplore.ieee.org/document/7696473 IEEE] | |||
|- | |||
| <html><la-tex>G_t</la-tex></html> || Transmitted gain || 832 || unitless || [https://ieeexplore.ieee.org/document/7696473 IEEE] | |||
|- | |||
| <html><la-tex>L_{atm}</la-tex></html> || Atmospheric loss || 1 || unitless || Assuming that at MHz frequencies, atmospheric loss is negligible | |||
|- | |||
| <html><la-tex>G_r</la-tex></html> || Receiver gain || <html><la-tex>6.6 \times 10^6</la-tex></html> || unitless || [https://descanso.jpl.nasa.gov/monograph/series13/DeepCommo_Chapter2--141029.pdf DESCANSO] (using DSN 34-m downlink gain) | |||
|- | |||
| <html><la-tex>k</la-tex></html> || Boltzmann Constant || <html><la-tex>1.38 \times 10^{-23}</la-tex></html> || J/K || Constant | |||
|- | |||
| <html><la-tex>T_{sys}</la-tex></html> || System temperature || 291 || K || [https://descanso.jpl.nasa.gov/DPSummary/DESCANSO18_MarCO.pdf DESCANSO] | |||
|- | |||
| <html><la-tex>R</la-tex></html> || Data Rate || <html><la-tex>8 \times 10^3</la-tex></html> || bps || [https://www.jpl.nasa.gov/news/press_kits/insight/launch/appendix/mars-cube-one/ JPL] | |||
|- | |||
| <html><la-tex>r</la-tex></html> || Distance from Earth (average) || <html><la-tex>2.28 \times 10^{11}</la-tex></html> || m || [https://science.nasa.gov/mars/facts/ NASA] | |||
|- | |||
| <html><la-tex>\lambda</la-tex></html> || Wavelength || 0.0356 || m || Conversion from 8.425 GHz | |||
|} | |||
We were curious about the sensitivity of power, transmitted gain, bit rate, and distance because these are all either design or mission architecture parameters that can be modified. The resulting partial derivatives are then: | |||
<html><la-tex>\frac{\partial \frac{E}{N}}{\partial P_t} = \frac{L_{\text{line}} G_t L_{\text{atm}} G_r}{k T_{\text{sys}} R} \left( \frac{4 \pi r}{\lambda} \right)^2</la-tex> | |||
<br> | |||
<br> | |||
<la-tex>\frac{\partial \frac{E}{N}}{\partial G_t} = \frac{P_t L_{\text{line}} L_{\text{atm}} G_r}{k T_{\text{sys}} R} \left( \frac{4 \pi r}{\lambda} \right)^2</la-tex> | |||
<br> | |||
<br> | |||
<la-tex>\frac{\partial \frac{E}{N}}{\partial R} = -\frac{P_t L_{\text{line}} G_t L_{\text{atm}} G_r}{k T_{\text{sys}} R} \left( \frac{4 \pi r}{R \lambda} \right)^2</la-tex> | |||
<br> | |||
<br> | |||
<la-tex>\frac{\partial \frac{E}{N}}{\partial r} = \frac{P_t L_{\text{line}} G_t L_{\text{atm}} G_r}{k T_{\text{sys}} R} \cdot 32 r \left( \frac{\pi}{\lambda} \right)^2</la-tex> | |||
<br> | |||
<br> | |||
</html> | |||
After plugging in values and normalizing, we get the following graph which tells us that a 1% improvement in transmitted power or transmitted gain will cause a 1% improvement in energy per bit noise spectral density. E/N is not very sensitive to bit rate or distance, as a 1% increase in bit rate would cause a 0.0012% decrease in E/N and a 1% increase in distance would lead to a 0.0024% increase in E/N. | |||
A tornado chart is shown below: <br> | |||
[[Image: EnergyBitPerNoiseTornado.png|800px]] | |||
<br> | |||
<br> | |||
'''Solar Array Efficiency''' | |||
Next, we performed a sensitivity analysis on solar array efficiency, the equation for which is given by: | |||
<html><la-tex>\eta = \frac{P4\pi r^2}{AL}*100</la-tex></html> | |||
<br> | |||
Where <html><la-tex>\eta</la-tex></html> is represented as a percent and the unknowns are listed and described below also for the MarCo satellite. | |||
{| class="wikitable" | |||
|+ Variables and Values | |||
|- | |||
! Variable !! Quantity Represented !! Value !! Unit !! Source | |||
|- | |||
| P || Power || 35 || W || [https://www.jpl.nasa.gov/news/press_kits/insight/launch/appendix/mars-cube-one/ (this time using Earth reference value)] | |||
|- | |||
| A || Area || 3.78 || m<sup>2</sup> || [https://www.eoportal.org/satellite-missions/marco#spacecraft] | |||
|- | |||
| r || Distance || 1.5 * 10<sup>11</sup> || m || 1 AU (distance from Sun to Earth used as a reference here) | |||
|- | |||
| L || Luminosity || 3.8 * 10<sup>26</sup> || W/m<sup>2</sup> || Constant | |||
|} | |||
The partial derivatives with respect to the parameters we can potentially alter are given as follows: | |||
<br><br> | |||
<html><la-tex>\frac{\partial \eta}{\partial P} = \frac{4\pi r^2}{AL}*100</la-tex> | |||
<br> | |||
<br> | |||
<la-tex>\frac{\partial \eta}{\partial A} = -\frac{P4\pi r^2}{A^2 L}*100</la-tex> | |||
<br> | |||
<br> | |||
<la-tex>\frac{\partial \eta}{\partial r} = \frac{P8\pi r}{AL}*100</la-tex> | |||
<br><br> | |||
</html> | |||
After plugging in values and normalizing, we get the following graph which tells us that a 1% improvement in power will cause a 1% improvement in solar efficiency, a 1% decrease in area will lead to a 1% increase in solar efficiency, and a 1% increase in distance correlates with a 0.02% increase in solar efficiency. | |||
A tornado chart is shown below: <br> | |||
[[Image: SolarEfficiencyTornado.png|800px]] | |||
===Morphological Matrix=== | ===Morphological Matrix=== | ||
{| class="wikitable" | |||
|- style="font-weight:bold; text-align:right; vertical-align:bottom;" | |||
! style="text-align:left;" | Subsystem | |||
! style="text-align:left;" | Component | |||
! style="text-align:left;" | Decision Variable | |||
! 1 | |||
! 2 | |||
! 3 | |||
! 4 | |||
|- | |||
| rowspan="4" | Communications | |||
| rowspan="4" | DSN-Compatible Radio | |||
| style="vertical-align:bottom;" | Type | |||
| style="vertical-align:bottom;" | Iris Radio | |||
| style="vertical-align:bottom;" | SCR-106 | |||
| style="vertical-align:bottom;" | SCR-108 | |||
| style="vertical-align:bottom;" | SWIFT-SLX | |||
|- style="text-align:right;" | |||
| style="vertical-align:bottom; text-align:left;" | Data rate [kbps] | |||
| style="vertical-align:bottom;" | 5313 | |||
| style="vertical-align:bottom;" | 20000 | |||
| style="vertical-align:bottom;" | 10000 | |||
| style="vertical-align:bottom;" | 6000 | |||
|- style="text-align:right;" | |||
| style="vertical-align:bottom; text-align:left;" | Transmitted Power [W] | |||
| style="vertical-align:bottom;" | 3 | |||
| style="vertical-align:bottom;" | 2.5 | |||
| style="vertical-align:bottom;" | 3 | |||
| style="vertical-align:bottom;" | 2 | |||
|- style="text-align:right;" | |||
| style="vertical-align:bottom; text-align:left;" | Mass [g] | |||
| style="vertical-align:bottom;" | 875 | |||
| style="vertical-align:bottom;" | 290 | |||
| style="vertical-align:bottom;" | 404 | |||
| style="vertical-align:bottom;" | 300 | |||
|- | |||
| rowspan="2" | Structure | |||
| rowspan="2" | Bus | |||
| style="vertical-align:bottom;" | Size | |||
| style="vertical-align:bottom;" | 3U | |||
| style="vertical-align:bottom;" | 6U | |||
| style="vertical-align:bottom;" | 12U | |||
| style="vertical-align:bottom;" | SmallSat | |||
|- | |||
| style="vertical-align:bottom;" | Maxmimum Mass | |||
| style="vertical-align:bottom;" | 6 kg | |||
| style="vertical-align:bottom;" | 14 kg | |||
| style="vertical-align:bottom;" | 25 kg | |||
| style="vertical-align:bottom;" | 250 kg | |||
|- | |||
| rowspan="2" | Power | |||
| rowspan="2" | Solar Panels | |||
| style="vertical-align:bottom;" | Cell Material | |||
| style="vertical-align:bottom;" | GaAs | |||
| style="vertical-align:bottom;" | ZTJ | |||
| style="vertical-align:bottom;" | CTJ30 | |||
| style="vertical-align:bottom;" | -- | |||
|- | |||
| style="vertical-align:bottom;" | Cell Efficiency | |||
| style="text-align:right; vertical-align:bottom;" | 30% | |||
| style="text-align:right; vertical-align:bottom;" | 29.50% | |||
| style="text-align:right; vertical-align:bottom;" | 29.50% | |||
| style="vertical-align:bottom;" | -- | |||
|- style="vertical-align:bottom;" | |||
| Propulsion | |||
| Propulsion System | |||
| Type | |||
| Cold gas | |||
| Ion | |||
| Propellant | |||
| Solar sail | |||
|- | |||
| rowspan="3" | GNC | |||
| style="vertical-align:bottom;" | Gyroscope | |||
| style="vertical-align:bottom;" | Type | |||
| style="vertical-align:bottom;" | Gyroscope | |||
| style="vertical-align:bottom;" | IMU | |||
| style="vertical-align:bottom;" | IRU | |||
| style="vertical-align:bottom;" | Magnetometers | |||
|- | |||
| style="vertical-align:bottom;" | Sun Sensors | |||
| style="vertical-align:bottom;" | Type | |||
| style="vertical-align:bottom;" | CSS | |||
| style="vertical-align:bottom;" | Cosine | |||
| style="vertical-align:bottom;" | Digital | |||
| style="vertical-align:bottom;" | Quadrant | |||
|- | |||
| style="vertical-align:bottom;" | Star Trackers | |||
| style="vertical-align:bottom;" | FOV [deg] | |||
| style="vertical-align:bottom;" | Small < 10 | |||
| style="vertical-align:bottom;" | Average < 15 | |||
| style="vertical-align:bottom;" | Large > 15 | |||
| style="vertical-align:bottom;" | -- | |||
|- style="vertical-align:bottom;" | |||
| Avionics | |||
| Memory Storage | |||
| Type | |||
| SRAM | |||
| DRAM | |||
| Flash | |||
| CRAM | |||
|} | |||
===Description of Tradespace=== | ===Description of Tradespace=== | ||
The above morphological matrix represents possible decision variables for certain components in a subset of subsystems to consider in the context of an interplanetary SmallSat. Specifically, the options for DSN-compatible radios are limited to only four: the Iris radio (Space Dynamics Laboratory), [https://innoflight.com/product/scr-106-scr-106hdr/ SCR-106] (Innoflight, Inc.), [https://innoflight.com/product/scr-108/ SCR-108] (Innoflight, Inc.), and [https://satsearch.co/products/tui-swift-slx SWIFT-SLX] (Tethers Unlimited). The smallest bus size for deep space is 3U, as planned with the [https://www.jpl.nasa.gov/missions/interplanetary-nano-spacecraft-pathfinder-in-relevant-environment-inspire/ INSPIRE mission]; the most popular choice is 6U, featured on the [https://www.space.com/nasa-artemis-1-moon-mission-cubesats Artemis 1 CubeSats]; the largest CubeSat variant is 12U, as seen on the [https://www.nasa.gov/smallspacecraft/capstone/ CAPSTONE mission]. Bus sizes beyond 12U are lumped into the SmallSat category, which includes missions such as Lunar Trailblazer and EscaPADES. The most common solar panel cells used in space missions are GaAs, ZTJ, and CTJ30, all of which have a cell efficiency of ~ 30%, which is the current state-of-the-art. Propulsion systems can vary wildly, as there is no standard, but attempted uses can be seen in MarCO's cold gas thrusters, [https://ieeexplore.ieee.org/abstract/document/9076195 Luna-H Map's] ion thrusters, and Lunar Flashlight's monopropellant system. Early versions of Lunar Flashlight and [https://science.nasa.gov/mission/nea-scout/ NEA Scout] included solar sail concepts. Interplanetary SmallSats require basic GNC systems, of which components come in a variety of standard forms. Finally, memory type is represented under Avionics in four industry standard options. | |||
==Key Publications and Patents== | ==Key Publications and Patents== | ||
===Publications=== | ===Publications=== | ||
The following publications feature future and past SmallSat missions beyond Earth. Links to each publication are provided, and a summary of the mission purpose and technical details is given. Notably, many of the lunar missions address the same science questions, and there is a definitive trend in components and suppliers. For example, BCT (Blue Canyon Technologies) produces state-of-the-art SmallSat guidance, navigation, and control components, JPL's Iris radio has seen multiple uses in-flight, and MMA design solar arrays are the current standard for solar power. | |||
<u>The Lunar Polar Hydrogen Mapper CubeSat Mission</u> | <u>The Lunar Polar Hydrogen Mapper CubeSat Mission</u> | ||
[https://ieeexplore.ieee.org/abstract/document/9076195 IEEE] | [https://ieeexplore.ieee.org/abstract/document/9076195 IEEE] | ||
Line 80: | Line 314: | ||
===Patents=== | ===Patents=== | ||
Though many SmallSat component designs are proprietary or open-source, the selected patents below highlight recent innovations in SmallSat technology. | |||
<u>SmallSat Platform with Standard Interfaces</u> | <u>SmallSat Platform with Standard Interfaces</u> | ||
Line 96: | Line 332: | ||
Propulsion system suitable for SmallSats and CubeSats. The basis is a hybrid solid rock motor including oxidizer tanks, nozzle, igniter, poly methyl methacrylate (PMMA) fuel, cold gas thrusters. | Propulsion system suitable for SmallSats and CubeSats. The basis is a hybrid solid rock motor including oxidizer tanks, nozzle, igniter, poly methyl methacrylate (PMMA) fuel, cold gas thrusters. | ||
To knowledge: not yet used in space | To knowledge: not yet used in space | ||
==Financial Model== | |||
[[File:RD_cost_savings.png|thumb|right|500px|alt=R&D Cost, Savings, and Discounted Cash Flow from 2022 through 2050|R&D Cost, Savings, and Discounted Cash Flow from 2022 through 2050]] | |||
[[File:Basiline_econ_outlook.png|thumb|right|500px|alt=Baseline Economic Outlook (NPV Analysis) showing Discounted Cash Flow and Cumulative Value|Baseline Economic Outlook (NPV Analysis) with Discounted Cash Flow and Cumulative Value]] | |||
The financial model for interplanetary small satellites highlights the economic feasibility of these technologies by examining R&D costs, savings/revenues, and discounted cash flow (DCF) over a 30-year timeline (2022–2050). | |||
The initial investment in R&D is set at $50 million/year in 2022, with costs declining exponentially at a rate of 10% per year as technology matures. By 2030, R&D costs are reduced to $20 million/year, and by 2050, they approach $5 million/year. | |||
Revenue begins ramping up in 2030, reaching $10 million/year, and continues to increase at a rate of $10 million/year until stabilizing at $200 million/year by 2049. | |||
This growth reflects the adoption of interplanetary small satellites for high-demand applications, such as deep-space communication, scientific exploration, and resource mining. | |||
DCF accounts for the time value of money using a 5% discount rate. Adjusted revenues begin to exceed adjusted costs by 2035, marking a breakeven point for the investment. | |||
From 2035 onward, the DCF grows steadily, reaching $50 million/year in net gains by 2040 and peaking at $150 million/year by 2050. | |||
Cumulative Discounted Cash Flow: | |||
Initially negative due to the high upfront costs, cumulative DCF crosses the $0 threshold by 2040, representing a net positive investment. | |||
By 2050, cumulative discounted gains exceed $2.5 billion, demonstrating the long-term economic viability of interplanetary small satellite programs. | |||
Insights from the Model | |||
Initial R&D Investments: Substantial early-stage investments (approximately $300 million in total by 2030) are required to achieve technology readiness levels (TRLs) suitable for interplanetary missions. These costs are justified by the exponential revenue growth projected after 2030. | |||
Revenue Growth: The model predicts savings/revenues of $200 million/year by 2050 due to increased adoption of interplanetary small satellites for communication, data relay, and mining. This aligns with current trends in the space economy, such as the rise of private sector participation and advancements in modular satellite designs. | |||
Breakeven Point: The breakeven point occurs in 2035, after which the investment becomes profitable. This is consistent with similar case studies in space technology, such as the economic performance of Earth observation satellites, which achieved profitability within 10–15 years of initial investment. | |||
Long-Term ROI: By 2050, the cumulative discounted cash flow exceeds $2.5 billion, delivering significant returns on the initial R&D investments. This long-term profitability supports NASA's Strategic Objectives 1.2 and 3.1, emphasizing the importance of space exploration technologies for science and innovation. | |||
Technical Implications | |||
R&D Optimization: The declining R&D costs reflect improvements in technology and manufacturing efficiency, which reduce the cost of individual satellite systems and components. | |||
Revenue Scaling: The rapid increase in savings/revenues corresponds to the scaling of interplanetary small satellites for diverse applications, including planetary science, asteroid mining, and deep-space communication. | |||
Economic Sustainability: The model demonstrates that interplanetary small satellites can achieve economic sustainability with modest initial investments compared to traditional spacecraft, making them an attractive option for public and private sectors alike. | |||
==Relevant, Ongoing R&D Projects== | |||
[https://techport.nasa.gov/projects/106836 Lightweight Integrated Solar Array and anTenna (LISA-T)]: a compact, thin film solar array that allows for 300% more power per mass and volume than current state-of-the-art arrays for smallsats. The prototype was launched about PTD-4 on August 16, 2024 to be demonstrated in microgravity, but the technology has direct applications to interplanetary missions. Currently at TRL-6. | |||
[https://techport.nasa.gov/projects/154434 RadPC]: this improved processor can accelerate key, computationally intensive mission tasks like navigation and science data processing. This technology has the potential to improve control and data handling for SmallSats. Earlier concepts have been demonstrated in Earth-orbiting satellites. Currently at TRL-4. | |||
[https://techport.nasa.gov/projects/154814 Ground Based Uplink and Beacon Laser for Long Range Communication]: a concept for a ground-based instrument that would enable optical communication to deep space satellites could revolutionize smallsat capabilities. Optical communication can supply data rates up to 100 times faster than radio frequencies. NASA hopes to demonstrate link length extending beyond 2AU. The Laser for Long Range Communication is currently at TRL-3. | |||
[https://techport.nasa.gov/projects/116432 Autonomous Satellite Technology for Resilient Application (ASTRA)]: a potentially game-changing advancement that could support more autonomous operations to small spacecraft. This technology is intended for demonstration during the Artemis missions with the technology being integrated into lunar-orbiting smallsats. It could transform the use of interplanetary smallsats away from just science and towards human mission operational support. Currently at TRL-7. | |||
==Technology Strategy Statement== | |||
Our strategy is to advance the use of SmallSats for missions beyond Earth's orbit. By emphasizing the use of commercial off-the-shelf components, CubeSat standardizations, and modular components, space exploration can be achieved with a smaller spacecraft at a smaller price. We begin with key technology demonstrations in Earth's orbit, expand to cislunar space to supplement the Artemis program, and use these experiences to establish a global constellation of science and communication CubeSats at Mars. The possibilities aren't limited here, as SmallSats have the potential to travel beyond Mars and to the inner solar system. Our goal is to advance SmallSat technology to expand the boundaries of human knowledge in an efficient and sustainable way. | |||
[[Image:4SMS_Technology_Swoosh_Chart.jpg|Technology swoosh chart for the future of interplanetary SmallSat exploration.]] |
Latest revision as of 20:55, 3 December 2024
Technology Roadmap Sections and Deliverables
Roadmap Overview
Interplanetary small satellites (SmallSats) are compact, lightweight spacecraft that are designed for science, exploration, and technology demonstration missions beyond Earth's orbit. These satellites, typically ranging in size from 3U-6U and up to 250 kg, leverage advancements in miniaturization, materials, and propulsion technology to conduct missions at a fraction of the cost of a traditional large spacecraft.
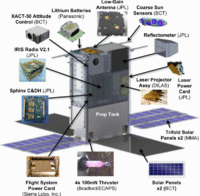
Small satellites-- including CubeSats, a specific type of SmallSat-- have traditionally been used in Low Earth Orbit (LEO) since the early 2000's. However, the key differences between interplanetary small satellites and Earth-observing small satellites arise from the need to function at distances much farther than LEO. One key difference is that current designs of interplanetary SmallSats require propulsion subsystems, a feature that is optional for Earth-orbiting SmallSats. Another difference is the method of communication. Whereas LEO satellites can communicate with simple RF ground stations nearly anywhere in the world, the infrastructure required to send and receive signals becomes increasingly complex with distance beyond LEO. Larger ground antennae are required to make up for signal weakening due to distance and free space path loss, which calls for the use of the Deep Space Network. Recent technological advancements, such as the creation of the Iris radio, have allowed these small spacecraft to take on interplanetary missions.
Unlike previous iterations of interplanetary spacecraft (Voyager, Cassini, Mars Reconnaissance Orbiter, etc.), SmallSats can accomplish similar feats to their predecessors for less. Examples of this technology include MarCo (twin 6U CubeSats) and Lunar Flashlight (6U lunar CubeSat), as well as upcoming missions such as Lunar Trailblazer (lunar SmallSat) and EscaPADE (Mars SmallSat twins). These satellites offer new opportunities for low-cost, high-impact science. NASA's SIMPLEx (Small, Innovative Missions for PLanetary Exploration) initiative anticipates this paradigm shift by supporting the formulation of future SmallSat missions. As technology continues to evolve, interplanetary SmallSats are expected to play an increasingly significant role in space exploration.
Design Structure Matrix (DSM) Allocation
Our DSM explores the uses and applications of different technologies within the autonomous space exploration taxonomy. We classified interplanetary small satellites within the orbiting spacecraft section of interplanetary autonomous spacecraft. The subsystems are listed within 4SMS, but could apply to most of the other technologies that stem from 2EOT or 2IPT.
In the top box, the higher level systems are categorized with regard to their applications. These are defense, science, and servicing. Defense refers to spacecraft that can be equipped for surveillance, intelligence, or weaponry. Science refers to spacecraft whose primary purpose is to collect and relay scientific information. Servicing includes spacecraft that support other preexisting missions or Earth-based systems such as Internet or GPS. We further explored what subsystems that are common to most spacecraft might support either of those three applications. Some boxes represent impossible connections; a spacecraft is either Earth-orbiting or interplanetary, it cannot be both. These intersections are highlighted in light gray.
The bottom box classifies the purpose and relationships of the subsystems that may make up an autonomous spacecraft. The three categories are information processing, transportation/navigation, and system support. If two subsystems do not have meaningful interaction, the box is left blank.
Legend:
- D – Defense Application
- C – Science Application
- V – Servicing Application
- I – Information Processing
- T – Transportation/Navigation
- S – System Support
- Grey box – Incompatible Connections
Roadmap Model using OPM
An example of a typical interplanetary SmallSat is shown in the Object-Process-Model (OPM) diagram below. The overall Object, Interplanetary SmallSat, is broken into Object blocks representing the various subsystems that comprise the SmallSat. Each subsystem is broken down even further to the component level. Component interactions are shown via the Process blocks and Relations arrows. The interactions between the SmallSat and the DSN, an external network, is also represented.
Figures of Merit (FOMs)
Data rate, power consumption, and mass are critical parameters for CubeSats, impacting mission success. Data rates for CubeSats have steadily improved from around 1200 bps in the 1990s to 10 Mbps today, mainly due to advances in RF transceivers, but are limited by the Shannon-Hartley theorem, which considers bandwidth and signal-to-noise ratio. Power consumption has also decreased from 5 watts in the 1990s to 3 watts in recent systems, thanks to energy-efficient designs, though CubeSats face constraints due to limited solar energy and battery storage. Mass reduction, achieved through miniaturization and material advances, has been modeled with a logistic function, showing rapid decreases in recent decades but approaching a theoretical limit due to essential components and operational durability in space.
A logistic function is used to model satellite mass reduction, where parameters include a theoretical minimum (L), midpoint year of rapid reduction (t₀), and growth rate (k). The curve indicates initial high mass, followed by rapid decreases, then a plateau as technological limits are approached.
Alignment with Company Strategic Drivers
Roughly every 3-5 years, NASA publishes a "Strategic Plan" intended to outline the key drivers behind their research, exploration, and discovery activities. The most recent version of this report is the NASA Strategic Plan 2022. Interplanetary SmallSats support Strategic Objectives 1.2 (Understand the Sun, solar system, and universe) and 3.1 (Innovate and advance transformational space technologies). Fundamentally, NASA cares about its ability to gain a deeper scientific understanding of its exploration destinations. While there is no direct mention of SmallSat technologies in the Strategic Plan, it is clear that they value both more scientific data and more remote destinations. As a result, uplink data rate and power efficiency will be the key FOMs to advance NASA's goals.
MarCO, the Mars CubeSat mission (2018), had spacecraft with power efficiency of 2.6 W/kg near Earth and 1.26 W/kg near Mars. This supported a data rate of 8 kilobytes per seconds (kbps) (source). The Lunar Flashlight project from a few years later nearly doubled the efficiency of MarCO's peak, operating at ~55W and only weighing 0.5 kg more than each MarCO satellite (source). Its efficiency at its end-of-life was about 3.93 W/kg. The uplink data rate, however, was the same as MarCO's: 8 kbps. Note that this is not a completely fair comparison, as Mars is usually over 500 times farther away than the Moon.
These two examples demonstrate that there is room for improvement in both FOM's.
We can look to Earth-orbiting satellites for examples of what is technologically feasible. Earth-orbiting large satellite readily achieve uplink data rates in the tens of megabytes range. State-of-the-art Earth-orbiting small satellites claim they can achieve somewhere in the hundreds of kbps. Power efficiency for Earth-orbiting small satellites typically produce around 10 W/kg. We believe these are ambitious, but achievable goals. Specifically, over the next decade as NASA prepares for increased human exploration activity, we believe it should aim to produce interplanetary SmallSats with uplink data rates in the ~50 kbps range and power efficiency in the 10 W/kg range.
Positioning of Company vs. Competition
The table below summarizes several notable interplanetary satellite missions and related efforts by NASA and other organizations to assess the competitive landscape for interplanetary small satellites. These missions are selected based on public data and illustrate the progression in uplink data rates, power efficiency, and payload capacities. They demonstrate evolving figures of merit (FOM) targets and underscore the unique challenges interplanetary satellites face compared to Earth-orbiting counterparts (NASA, 2022; JPL, 2018).
Interplanetary small satellites often face steep requirements in power efficiency and data transmission compared to Earth-orbiting satellites, as shown by comparing MarCO’s low data rate with that of typical Earth-orbiting satellites achieving over 100 kbps. In recent projects like Lunar Flashlight and Lunar Trailblazer, we see improvements in power efficiency, with Flashlight achieving nearly double MarCO’s peak efficiency. However, most missions struggle with maintaining high data rates across vast distances, given the limitations in miniaturized radio technology for deep space (NASA, 2022; ESA, 2020).
Competitive FOM targets demonstrate that despite growing advancements, NASA’s interplanetary small satellites lag behind some Earth-orbiting satellites in terms of uplink data rates and power efficiency. While Earth-orbiting satellites can reach power efficiencies of 10 W/kg, interplanetary missions rarely achieve more than 4-5 W/kg, and uplink rates remain around 10 kbps or lower (ESA, 2020). As illustrated in the table, other space agencies, such as ESA, are pursuing interplanetary small satellites with similar limitations. This competitive analysis highlights how constraints unique to deep space missions slow the attainment of ambitious FOM targets seen in NASA’s Strategic Objective 3.1.
Technical Model
Sensitivity Analysis
Energy Bit per Noise Spectral Density
The first equation we analyzed is the energy bit per noise spectral density given by:
Where
Variable | Quantity Represented | Value | Unit | Source |
---|---|---|---|---|
Power transmitted | 17 | W | JPL | |
Line loss | 0.6966 | unitless | IEEE | |
Transmitted gain | 832 | unitless | IEEE | |
Atmospheric loss | 1 | unitless | Assuming that at MHz frequencies, atmospheric loss is negligible | |
Receiver gain | unitless | DESCANSO (using DSN 34-m downlink gain) | ||
Boltzmann Constant | J/K | Constant | ||
System temperature | 291 | K | DESCANSO | |
Data Rate | bps | JPL | ||
Distance from Earth (average) | m | NASA | ||
Wavelength | 0.0356 | m | Conversion from 8.425 GHz |
We were curious about the sensitivity of power, transmitted gain, bit rate, and distance because these are all either design or mission architecture parameters that can be modified. The resulting partial derivatives are then:
After plugging in values and normalizing, we get the following graph which tells us that a 1% improvement in transmitted power or transmitted gain will cause a 1% improvement in energy per bit noise spectral density. E/N is not very sensitive to bit rate or distance, as a 1% increase in bit rate would cause a 0.0012% decrease in E/N and a 1% increase in distance would lead to a 0.0024% increase in E/N.
A tornado chart is shown below:
Solar Array Efficiency
Next, we performed a sensitivity analysis on solar array efficiency, the equation for which is given by:
Where
Variable | Quantity Represented | Value | Unit | Source |
---|---|---|---|---|
P | Power | 35 | W | (this time using Earth reference value) |
A | Area | 3.78 | m2 | [1] |
r | Distance | 1.5 * 1011 | m | 1 AU (distance from Sun to Earth used as a reference here) |
L | Luminosity | 3.8 * 1026 | W/m2 | Constant |
The partial derivatives with respect to the parameters we can potentially alter are given as follows:
After plugging in values and normalizing, we get the following graph which tells us that a 1% improvement in power will cause a 1% improvement in solar efficiency, a 1% decrease in area will lead to a 1% increase in solar efficiency, and a 1% increase in distance correlates with a 0.02% increase in solar efficiency.
A tornado chart is shown below:
Morphological Matrix
Subsystem | Component | Decision Variable | 1 | 2 | 3 | 4 |
---|---|---|---|---|---|---|
Communications | DSN-Compatible Radio | Type | Iris Radio | SCR-106 | SCR-108 | SWIFT-SLX |
Data rate [kbps] | 5313 | 20000 | 10000 | 6000 | ||
Transmitted Power [W] | 3 | 2.5 | 3 | 2 | ||
Mass [g] | 875 | 290 | 404 | 300 | ||
Structure | Bus | Size | 3U | 6U | 12U | SmallSat |
Maxmimum Mass | 6 kg | 14 kg | 25 kg | 250 kg | ||
Power | Solar Panels | Cell Material | GaAs | ZTJ | CTJ30 | -- |
Cell Efficiency | 30% | 29.50% | 29.50% | -- | ||
Propulsion | Propulsion System | Type | Cold gas | Ion | Propellant | Solar sail |
GNC | Gyroscope | Type | Gyroscope | IMU | IRU | Magnetometers |
Sun Sensors | Type | CSS | Cosine | Digital | Quadrant | |
Star Trackers | FOV [deg] | Small < 10 | Average < 15 | Large > 15 | -- | |
Avionics | Memory Storage | Type | SRAM | DRAM | Flash | CRAM |
Description of Tradespace
The above morphological matrix represents possible decision variables for certain components in a subset of subsystems to consider in the context of an interplanetary SmallSat. Specifically, the options for DSN-compatible radios are limited to only four: the Iris radio (Space Dynamics Laboratory), SCR-106 (Innoflight, Inc.), SCR-108 (Innoflight, Inc.), and SWIFT-SLX (Tethers Unlimited). The smallest bus size for deep space is 3U, as planned with the INSPIRE mission; the most popular choice is 6U, featured on the Artemis 1 CubeSats; the largest CubeSat variant is 12U, as seen on the CAPSTONE mission. Bus sizes beyond 12U are lumped into the SmallSat category, which includes missions such as Lunar Trailblazer and EscaPADES. The most common solar panel cells used in space missions are GaAs, ZTJ, and CTJ30, all of which have a cell efficiency of ~ 30%, which is the current state-of-the-art. Propulsion systems can vary wildly, as there is no standard, but attempted uses can be seen in MarCO's cold gas thrusters, Luna-H Map's ion thrusters, and Lunar Flashlight's monopropellant system. Early versions of Lunar Flashlight and NEA Scout included solar sail concepts. Interplanetary SmallSats require basic GNC systems, of which components come in a variety of standard forms. Finally, memory type is represented under Avionics in four industry standard options.
Key Publications and Patents
Publications
The following publications feature future and past SmallSat missions beyond Earth. Links to each publication are provided, and a summary of the mission purpose and technical details is given. Notably, many of the lunar missions address the same science questions, and there is a definitive trend in components and suppliers. For example, BCT (Blue Canyon Technologies) produces state-of-the-art SmallSat guidance, navigation, and control components, JPL's Iris radio has seen multiple uses in-flight, and MMA design solar arrays are the current standard for solar power.
The Lunar Polar Hydrogen Mapper CubeSat Mission IEEE
We do not currently understand the concentration of water ice volatiles at the South Pole of the Moon. Luna-H Map is a 6U CubeSat that will pass over the South Pole with a neutron spectrometer (Mini-NS) to map hydrogen concentrations. Components include: Mini-NS (payload), Iris radio, BIT-3 ion thruster (propulsion), MMA Design HaWK and eHaWK solar arrays (power), BCT XBI-50 (avionics and GNC).
Lunar Flashlight: Illuminating the Lunar South Pole IEEE
We do not currently understand the composition, quantity, distribution, and form of water and other volatiles at the South Pole of the Moon. Lunar Flashlight is a 6U CubeSat that will pass over the South Pole with an infrared spectrometer to determine concentrations of water ice on the Moon. Components include: BCT XACT-50 (attitude control), Sphinx using F Prime (avionics), Iris radio, 4x 100mN ASCENT thrusters (propulsion), trifold MMA Design High Watts per Kilogram solar arrays and 2Ux3U solar arrays.
NASA’s Lunar Trailblazer Mission: A Pioneering Small Satellite for Lunar Water and Lunar Geology IEEE
We do not understand the form, abundance, and distribution of water in the South pole, nor do we have detailed maps of surface temperatures. Lunar Trailblazer is a science-driven SmallSat. Components include: HVM^3 High-resolution volatiles and minerals Moon mapper imaging spectrometer (payload), LTM Lunar Thermal Mapper thermal infrared multispectral imager (payload), monoprop hydrazine system (propulsion), Iris radio, Sphinx (avionics).
MarCO: Interplanetary Mission Development on a CubeSat Scale Springer
MarCO is a twin 6U CubeSat mission that launched with the Insight lander. Its purpose was to relay data from the Insight lander to the DSN to account for a MEP coverage gap during Insight’s early operations. Components include: Iris radio, MMA Design LLC 3U solar arrays, BCT XACT (ACS), cold gas propulsion, cameras.
CAPSTONE: A CubeSat Pathfinder for the Lunar Gateway Ecosystem Small Satellite Conference
CAPSTONE is a 12U CubeSat mission whose purpose is to demonstrate a near-rectilinear halo orbit that will be used for the Lunar Gateway and to demonstrate the use of the Cislunar Autonomous Positioning Systems. Components include: Tyvak 12U bus, CAPS (payload), monopropellant hydrazine thrusters.
Patents
Though many SmallSat component designs are proprietary or open-source, the selected patents below highlight recent innovations in SmallSat technology.
SmallSat Platform with Standard Interfaces
CPC: B64G 1/10, B64G 1/428, and B64G 1/641
Cylindrical SmallSat platform (bus) design with a pre-determined electrical and mechanical interfaces including a payload adapter ring. “CapSat” alternative to the traditional CubeSat; variety of sizes included.
SmallSat Hybrid Propulsion System
CPC: F02K 9/32, F02K 9/80, and F02K 9/95
Propulsion system suitable for SmallSats and CubeSats. The basis is a hybrid solid rock motor including oxidizer tanks, nozzle, igniter, poly methyl methacrylate (PMMA) fuel, cold gas thrusters. To knowledge: not yet used in space
Financial Model
The financial model for interplanetary small satellites highlights the economic feasibility of these technologies by examining R&D costs, savings/revenues, and discounted cash flow (DCF) over a 30-year timeline (2022–2050).
The initial investment in R&D is set at $50 million/year in 2022, with costs declining exponentially at a rate of 10% per year as technology matures. By 2030, R&D costs are reduced to $20 million/year, and by 2050, they approach $5 million/year.
Revenue begins ramping up in 2030, reaching $10 million/year, and continues to increase at a rate of $10 million/year until stabilizing at $200 million/year by 2049. This growth reflects the adoption of interplanetary small satellites for high-demand applications, such as deep-space communication, scientific exploration, and resource mining.
DCF accounts for the time value of money using a 5% discount rate. Adjusted revenues begin to exceed adjusted costs by 2035, marking a breakeven point for the investment. From 2035 onward, the DCF grows steadily, reaching $50 million/year in net gains by 2040 and peaking at $150 million/year by 2050.
Cumulative Discounted Cash Flow: Initially negative due to the high upfront costs, cumulative DCF crosses the $0 threshold by 2040, representing a net positive investment. By 2050, cumulative discounted gains exceed $2.5 billion, demonstrating the long-term economic viability of interplanetary small satellite programs. Insights from the Model Initial R&D Investments: Substantial early-stage investments (approximately $300 million in total by 2030) are required to achieve technology readiness levels (TRLs) suitable for interplanetary missions. These costs are justified by the exponential revenue growth projected after 2030.
Revenue Growth: The model predicts savings/revenues of $200 million/year by 2050 due to increased adoption of interplanetary small satellites for communication, data relay, and mining. This aligns with current trends in the space economy, such as the rise of private sector participation and advancements in modular satellite designs.
Breakeven Point: The breakeven point occurs in 2035, after which the investment becomes profitable. This is consistent with similar case studies in space technology, such as the economic performance of Earth observation satellites, which achieved profitability within 10–15 years of initial investment.
Long-Term ROI: By 2050, the cumulative discounted cash flow exceeds $2.5 billion, delivering significant returns on the initial R&D investments. This long-term profitability supports NASA's Strategic Objectives 1.2 and 3.1, emphasizing the importance of space exploration technologies for science and innovation.
Technical Implications R&D Optimization: The declining R&D costs reflect improvements in technology and manufacturing efficiency, which reduce the cost of individual satellite systems and components. Revenue Scaling: The rapid increase in savings/revenues corresponds to the scaling of interplanetary small satellites for diverse applications, including planetary science, asteroid mining, and deep-space communication. Economic Sustainability: The model demonstrates that interplanetary small satellites can achieve economic sustainability with modest initial investments compared to traditional spacecraft, making them an attractive option for public and private sectors alike.
Relevant, Ongoing R&D Projects
Lightweight Integrated Solar Array and anTenna (LISA-T): a compact, thin film solar array that allows for 300% more power per mass and volume than current state-of-the-art arrays for smallsats. The prototype was launched about PTD-4 on August 16, 2024 to be demonstrated in microgravity, but the technology has direct applications to interplanetary missions. Currently at TRL-6.
RadPC: this improved processor can accelerate key, computationally intensive mission tasks like navigation and science data processing. This technology has the potential to improve control and data handling for SmallSats. Earlier concepts have been demonstrated in Earth-orbiting satellites. Currently at TRL-4.
Ground Based Uplink and Beacon Laser for Long Range Communication: a concept for a ground-based instrument that would enable optical communication to deep space satellites could revolutionize smallsat capabilities. Optical communication can supply data rates up to 100 times faster than radio frequencies. NASA hopes to demonstrate link length extending beyond 2AU. The Laser for Long Range Communication is currently at TRL-3.
Autonomous Satellite Technology for Resilient Application (ASTRA): a potentially game-changing advancement that could support more autonomous operations to small spacecraft. This technology is intended for demonstration during the Artemis missions with the technology being integrated into lunar-orbiting smallsats. It could transform the use of interplanetary smallsats away from just science and towards human mission operational support. Currently at TRL-7.
Technology Strategy Statement
Our strategy is to advance the use of SmallSats for missions beyond Earth's orbit. By emphasizing the use of commercial off-the-shelf components, CubeSat standardizations, and modular components, space exploration can be achieved with a smaller spacecraft at a smaller price. We begin with key technology demonstrations in Earth's orbit, expand to cislunar space to supplement the Artemis program, and use these experiences to establish a global constellation of science and communication CubeSats at Mars. The possibilities aren't limited here, as SmallSats have the potential to travel beyond Mars and to the inner solar system. Our goal is to advance SmallSat technology to expand the boundaries of human knowledge in an efficient and sustainable way.